Abstract
Creatine (Cr) and its analog phosphocreatine (PCr) are naturally occurring compounds found in human tissues. Both compounds play an important role in energy metabolism in skeletal muscle through participation in the creatine kinase (CK) reaction.
Cr is produced in the liver by enzymatic transmethylation of guanidinoacetic acid (GAA) from the kidneys. It is transported to muscle cells through the blood and taken up against a large concentration gradient by action of the creatine transporter (CreaT).
In skeletal muscle tissue, Cr is converted to PCr through the CK reaction. This allows inorganic phosphate to be stored at sites of ATP consumption for immediate regeneration of ATP from ADP. In addition to its role in energy metabolism, Cr also affects protein synthesis and mitigates skeletal muscle tissue damage.
Cr degrades spontaneously through a process of nonenzymatic conversion into creatinine (Crn). This occurs at a steady rate. Adequate Cr levels in the cell are maintained by endogenous synthesis or absorption following ingestion.
Introduction
Creatine (Cr) was discovered as an ingredient in meat in 1832 by the French scientist Chevreul, who named it after the Greek word kreas, meaning “flesh”. The Cr analog phosphocreatine (PCr) was discovered in 1927, followed by the discovery of the creatine kinase (CK) reaction in 1937. In the years following, most research on the subject focused on various biochemical aspects of the CK reaction and its role in tissues with high energy demands. (1)
At the start of the 1990s, Cr received widespread attention for its use as an ergogenic aid. This followed public knowledge of its use by British track and field athletes in training for the 1988 and1992 summer Olympic Games. The British experienced considerable success in these games, and many people wondered whether this success was aided by Cr supplementation.
Much of the ensuing controversy arose over how to categorize creatine. Those who asserted that it should be prohibited from use by Olympic athletes believed that it lended an unfair—and more importantly, unnatural—advantage, much like steroids. On the other hand, others observed that Cr is found in certain foods containing it (meat) and contested that it should therefore be permitted, like protein or vitamin supplements.
The polemics surrounding this dispute created a sensation, and many were quick to capitalize off of it. Cr began to be marketed to consumers as an athletic nutritional supplement. Much has been written on the subject of Cr use, and a large number of clinical and laboratory studies have been performed to better understand the compound. In recent years, research on the subject has shifted from performance evaluation to studies elucidating the role of Cr in muscle functional capacity.
Presently, Cr is a popularly known substance, and is available as a nutritional supplement in most health-food and dietary-supplement stores. It is a commonly used consumer product, mostly by young adults who wish to increase their athletic performance. As stated earlier, much has already been written on the subject of Cr use. However, in the lay literature, it is difficult to find a clear, concise and adequately informative chemical description of Cr as it functions in human muscle tissue. The intent of this paper is to provide a comprehensive overview of the synthesis of Cr in the human body and its function in human muscle tissue.
Creatine Synthesis
Cr is a small molecule resembling an amino acid, such as arginine or lysine. It cooperates with CK to buffer the ATP pool in muscle tissue. It is naturally found in the body, with approximately 95% of the body's total Cr pool (tCr, the amount of Cr in both phosphorylated and non-phosphorylated forms) being concentrated in skeletal muscle tissue, with smaller amounts being found in the brain, heart and, in males, the testes. Cr can either be obtained through the diet by eating the skeletal muscles of other animals, or produced endogenously by the body. (1)
GAA Synthesis
Cr is synthesized through a multi-step process in different areas of the body and then transported to tissues where it is utilized. This synthesis process begins in the kidneys with the formation of guanidinoacetic acid (GAA) from arginine and glycine. The reaction is catalyzed by arginine:glycine amidinotransferase (AGAT), and produces ornithine as a by-product (Figure 1). This reaction is the rate-limiting step in Cr synthesis. After formation, GAA is transported in the blood from the kidney to the liver. (2)
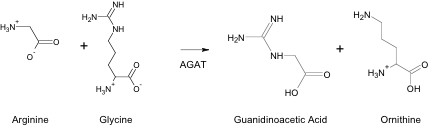
Cr Synthesis from GAA
In the liver, GAA is methylated by S-adenosylmethionine (SAMe), which is the methyl donor in virtually all known biological methylations (Figure 2). The reaction is catalyzed by Guanidino-adenosyl methyltransferase (GAMT). This enzymatic transmethylation reaction forms Cr and S-adenosylhomocystine (SAHe), and consumes more SAMe than all other methylation reactions in the body combined.
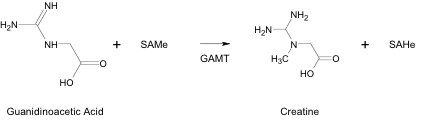
SAMe contains a methyl group attached to a positive-charged sulfur atom. The positive charge pulls electron density away from the methyl group, allowing it to be detached as a carbenium ion equivalent and transferred to an ion having a lone pair of electrons, such as the nitrogen atom in GAA. This reaction is catalyzed by guanidinoacetate methyltransferase (GAMT). Upon formation, Cr is released into the bloodstream and made available for muscle tissue uptake. (2)
Muscle Tissue Uptake
Approximately 90% of cellular Cr is taken up and accumulated against a large concentration gradient. This is facilitated by the Cr transporter (CreaT), which is driven by electrochemical potential differences of extracellular vs. intracellular sodium and chloride ion concentrations. (3)
CreaT is a member of the sodium ion-dependent neurotransmitter transporter family, closely related to those for dopamine, guanidino g -aminobutyric acid and taurine. The gene for CreaT is located on chromosome Xq28 and is labeled the Cr transporter gene (CreaT1). Its coding sequence is located by Genbank accession number L31409. It consists of 3747 base pairs (bp), with the encoding region beginning at bp 474. It contains two poly-A tails beginning at nucleotides 2550 and 3666. (4)
The coding region on CreaT1 contains 13 exons. The first eight cover transmembrane domains. Further afield, the intron/exon structures become more compact and the transmembrane domains span the intron/exon boundaries. (4)
The CreaT protein contains 635 amino acids, with 12 transmembrane spanning domains. The most variable regions between CreaT and other members of the same family occur in the 9 th to 11 th transmembrane domains, as well as at both ends of the protein. (4)
Recently, a second CreaT gene (CreaT2) has been discovered on chromosome 16p11.1-16p11.2. However, mRNA transcripts from this gene may only be expressed in the testes, which also express mRNA from the Xq28 locus, as well. Both CreaT1 and CreaT2 display 97% homology. The functional significance of this is unclear. Researchers have reported findings that CreaT2 identifies a premature stop codon within exon 4, encoding a non-functional or truncated protein. Other researchers have found that the CreaT2 protein contains 50 additional amino acids compared to that from CreaT1. These conflicting results, however, may be explainable by the fact that each research group used different coding sequences in obtaining their data. (4)
The CreaT protein is localized within the plasma membrane of skeletal muscle cells and mitochondria. Although type-II skeletal muscle tissue has been shown to have a higher total Cr concentration compared to type-I, there has not been any observed difference in CreaT content between the two fiber types. (3)
CreaT may be glycosylated and phosphorylated. These functions may be important in the regulation of its activity. It is believed that CreaT contains 3 phosphorylation sites, some of which may be phosphorylated by cAMP-dependent protein kinase A. The remainder are believed to be phosphorylated by protein kinase C. (4) In addition to the phosphorylation sites, CreaT is also believed to contain 5 glycosylation sites. CreaT activity is also known to be affected by factors such as catacholemines, insulin-like growth factor 1, insulin and exercise. (5)
Creatine Function in Skeletal Muscle Tissue
Within skeletal muscle tissue, Cr reacts with ATP to form PCr, ADP and a hydrogen ion. This reaction is reversible and can proceed in either direction, depending on the immediate need for ATP within the muscle cell (Figure 3).
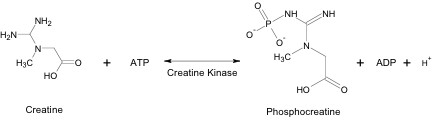
Creatine Kinase
Cr function is contingent on CK. This enzyme is usually specifically localized within the cell at sites of ATP production and consumption. At sites of ATP production (such as the mitochondria), it acts in building up a PCr pool in the cell. At sites of ATP consumption, CK functions in restoring ATP.
CK displays a number of isoenzymes in skeletal muscle tissue. These isoenzymes can be divided into two categories: cytosolic muscle-type CK (MM-CK) and mitochondrial CK (Mt-CK). In general, Mt-CK exists as two isoenzymes throughout the body. The ubiquitous form (uMt-CK) is found in various tissues such as the brain and the kidneys, while the sarcomeric form (sMt-CK) is restricted to striated muscle. Both of these mitochondrial isoenzymes have been studied, and have been found to display different biochemical, kinetic and structural properties. (7)
Mechanism of CK
The most likely mechanism for the phosphorylation of Cr from ATP involves a highly reactive metaphosphate intermediate. This compound is generated in the rate-determining step of the CK reaction by a proton transfer from CK to PCr, and cleavage of the P—N bond of PCr. (8) This process occurs in one step, and is the most important contribution of CK to the overall reaction.
Cr Role in Energy Metabolism
In Cr-containing tissues, and skeletal muscle tissue in particular, Cr plays an important role in energy metabolism. Through the CK reaction, Cr acts as both a temporal energy and pH buffer. This reaction catalyzes the reversible transfer of the g -phosphate group of ATP to the guanidino group of Cr to yield ADP and PCr. Through this process, muscle cells are able to build up a store of inorganic phosphate in the form of PCr for the use of immediate ATP generation in periods of acute need. This process can be sustained for up to 10 seconds in healthy individuals, and provides space until the body can begin to generate ATP through glycolytic and oxidative pathways. (9)
The CK reaction is pH dependent, favoring the formation of ATP and Cr in acidic conditions, such as that present when the muscle is performing intense work. ATP formation from ADP though the CK reaction consumes hydrogen ions, thus acting to decrease the pH in the cell. At higher pH levels, such as those present when muscle is at rest, the CK reaction favors the formation of PCr and ADP. This functions in locking Cr into the cell in the form of PCr and retaining it for later use. (5)
Cr and PCr also play a role in glycolysis regulation through dynamic interaction with oxidative enzymes within the cell. Skeletal muscle contains sensitive biochemical controls regulating various metabolic pathways, and PCr levels within cells can be a factor in the activity of mitochondrial CK, succinate dehydrogenase, citrate synthase and GLUT-4 glucose transporters. PCr levels can also inhibit glycogen phosphorylase, phosphofructokinase, pyruvate kinase and lactate dehydrogenase.
Cr Effects on Protein Synthesis
Dietary Cr supplementation in healthy individuals can have the beneficial effect of enhanced muscle size and increased lean-body mass. Studies have investigated this and found that Cr and PCr have a marked effect on protein synthesis within the skeletal muscle cell. When Cr accumulates within a cell, water drag occurs causing hyperhydration, which acts as an anabolic signal and stimulates protein synthesis. In the opposite situation, hypo-osmality acts as a signal to reduce protein degradation. The presence of Cr in skeletal muscle cells may also stimulate satellite cell miotic activity and possibly decrease protein catabolism. (10)
Cr Role in Tissue Damage Prevention
PCr functions in tissue damage prevention by stabilizing cellular membranes and maintaining ATP. The zwitterion character of PCr, which has a negatively charged phosphate group and a positively charged guanidino group, causes it to bind to the phospholipid head groups on the cellular membrane. This decreases membrane fluidity and loss of cytoplasmic contents from the cell. PCr further prevents tissue damage by allowing for a higher reserve of ATP. This temporal buffer, as described earlier, prevents tissue damage that would occur were conditions otherwise, such as in cases of transient ischemia. (7)
Cr Degradation
Creatine spontaneously degrades into creatinine (Crn) at a rate of approximately 1.6% of total Cr content per day. This is an irreversible process that takes place through a nonenzymatic conversion (Figure 4). A small portion of Crn may be converted into Arg or guanidinobutyrate. Crn is membrane permeable and it diffuses out of tissues. It is excreted by the kidneys into urine by glomular filtration. The constant loss of Cr in this manner is compensated for by healthy individuals through endogenous synthesis or ingestion of Cr in the diet. (1)
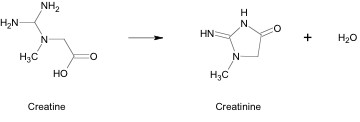
Conclusion
Cr and PCr are important assets/include of the process of energy metabolism through participation in the CK reaction. Both compounds are naturally produced in the body through a multi-step process in the kidneys and the liver. Cr is accumulated in skeletal muscle tissue against a large concentration gradient, and functions to immediately restore ATP in the cell. Cr and PCr also affect protein synthesis and play a role in tissue damage prevention. Cr spontaneously degrades to form Crn, which is excreted by the body.
Literature Cited
- Wyss, M.; Kaddurah-Daouk, R. Creatine and creatinine metabolism. Phys. Rev. 2002 , 80 (3), 1107–1182.
- Nelson, David L. and Michael M. Cox; Lehninger Principles of Biochemistry; Third Edition; Worth Publishers: New York, 2000; pp. 502, 510–11, 631, 842, 874–75.
- Walzel, B.; Speer, O.; Boehm, E.; Kristiansen, S.; Chan, S.; Clarke, K.; Magyar, J.; Richter, E.; Wallimann, T. New creatine transporter assay and identification of distinct creatine transporter isoforms in muscle. Am. J. Physiol. Endocrinol. Metab. 2002, 283, E390–E401.
- Snow, R.; Murphy, R. Creatine and the creatine transporter: a review. Mol. Cell. Biochem. 2001, 224, 169–181.
- Persky, A.; Brazeau, G. Clinical pharmacology of the dietary supplement creatine monohydrate. Pharm. Rev., 2001, 53, 161–176.
- Schlattner, U.; Eder, M.; Dolder, M.; Khucha, Z.; Strauss, A.; Wallimann, T. Divergent enzyme kinetics and structural properties of the two human mitochondrial creatine kinase isoenzymes. Biol. Chem. 2000, 381, 1063–1070.
- Greenhaff, P.; The nutritional biochemistry of creatine. Nutr. Biochem., 1997, 8, 610–618.
- Haake, P.; Allen, G. Studies on phosphorylation by phosphoguanidates. The mechanism of action of creatine: ATP transphosphorylase (Creatine Kinase). Proc. Nat. Acad. Sci. USA, 1971, 68, 2691–2693.
- de Groof, A.; Fransen, J. ; Errington, R. ; Willems, P.; Wieringa, B.; Koopman, W. The creatine kinase system is essential for optimal refill of the sarcoplasmic reticulum Ca2+ store in skeletal muscle. J. Biol. Chem., 2002, 277, 5275–5284.
- Hespel, P.; Op't Eijnde, B.; Derave, W.; Richter, E. Creatine supplementation: Exploring the role of the creatine kinase/phosphocreatine system in human muscle. Can. J. Appl. Phys., 2001, 26, S79–S102.